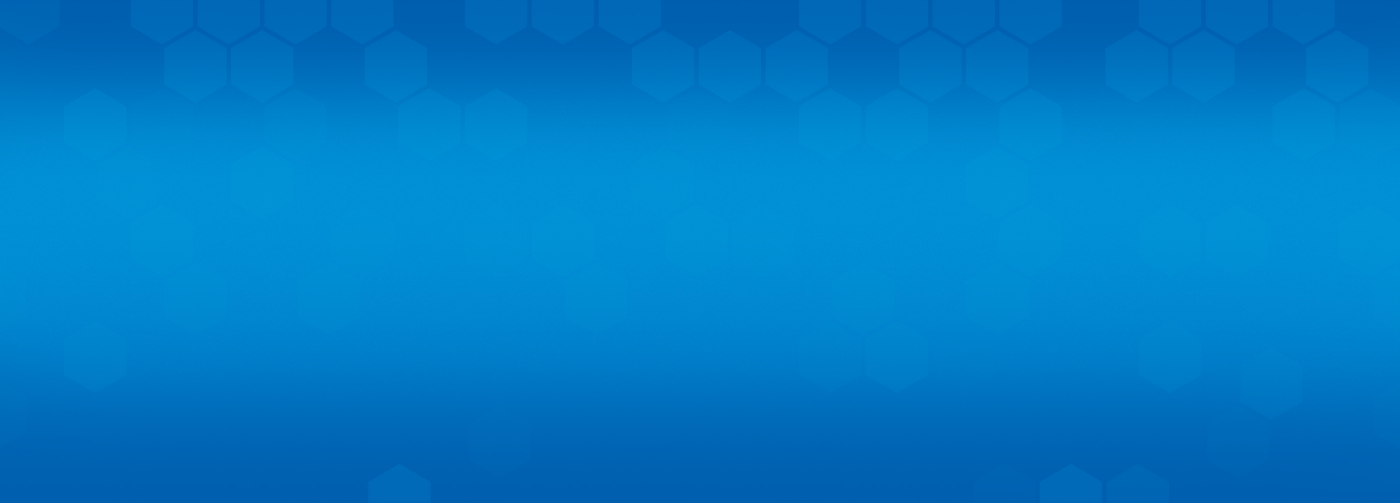
Life Science Optics
FPs have greatly expanded the scientific fluorescence toolbox, thereby enabling new capabilities. At the same time, they also have brought in new aspects to consider. When deciding to use fluorescent proteins in an application, what are the factors that should be taken into account?
It is important to keep in mind that most FP applications involve microscopy, with the wavelengths of interest stretching from the ultraviolet to the infrared. Thus, accessories impacting microscopy imaging are important. For most applications, the emphasis is on the visible. There is, however, an increasing interest in the far red, largely because these photons are less energetic and therefore cause less cellular damage.
Specific applications place different demands. In live cell imaging, for instance, one frequent application is the observation of cellular dynamics. Capturing fast events requires rapid image acquisition, which in turn demands short exposure times. Consequently, the optical system should include high sensitivity detectors and a suitably bright light source, as well as objectives with the right numerical aperture and other characteristics.
The best filter set to use is one optimized for high throughput. Key parameters include very steep spectral edges and high out-of-band blocking, thereby allowing as much of the desired light through while keeping out unwanted stray light. Also, the filters typically need high transmission (%T), which brings important benefits. FPs have to be excited into fluorescence, which means that live cells have to be exposed to excitation light. However, intense excitation light can damage the cells through phototoxicity and can increase the rate of photobleaching. The first damages or kills the cells while the second destroys the FP signal. High %T filters allow more excitation light in and permit better collection efficiency of the emission. As a result, they minimize phototoxicity and photobleaching, and so can enhance live cell imaging.
The fact that live cells are the focus of these applications has other implications for optical components. Typically, cell samples have to be maintained at 37° C, often at a controlled CO2 concentration and humidity. These environmental conditions may be achieved using an enclosure, and any optical filters might be inside the enclosure. In these circumstances, the best choice is likely to be hard-coated filters. They do not degrade or suffer damage as a result of these environmental conditions. Soft-coated filters, on the other hand, can be damaged. In general, in any situation, the reliability of the filters and other optical components is important.
For optical highlighter applications, there are additional considerations. They typically require two distinct excitation bands, one for photoconversion and another for excitation. Both travel over at least some of the same optical path, as does the outgoing emission. Therefore, dichroic beamsplitters and associated filters have to be used. The optics must have wide reflection bands, including reflection in the UV. The latter is needed because photoconversion often requires a UV source.
The challenge is that high energy UV illumination can create cellular damage, so care has to be taken to optimize the illumination intensity. One way to do so is via neutral density filters. Another is to reduce the exposure time. Because of overlapping optical paths, filters in the excitation path need to have good blocking within the passband of any emission wavelengths.
Using optical highlighters to achieve super-resolution places its own demands on optical components. Beating the diffraction limit demands what are effectively multiple passes. Thus, like live cell imaging, this application benefits from high %T filters and the resulting increase in throughput.
In the case of multicolor imaging, there is a need to separate out FP signals. A striking and scientifically important example can be found in the so-called Brainbow mice created by Harvard researchers Jean Livet and Jeff Lichtman in 2007. Now available commercially, these transgenic mice have randomly arranged fluorescent proteins in their neurons. Because the integration sites have multiple copies of the transgene, each neuron may express one of many possible FP combinations and distinct hues. There may be 150+ distinguishable colors, with the expression and resulting color being modified by the use of a promoter.
(Photo used with permission from Jeffrey Lichtman, MD, Professor of Molecular and Cellular Biology, Harvard University, Cambridge MA)
In designing the transgenes, the researchers created one with a membrane tether to allow axonal processes to be labeled, another with a nuclear localization signal, and a third that is distributed throughout the cytoplasm. These mice could allow researchers to map the circuits of the brain. However, investigators will have to differentiate between signals in what looks like multicolored abstract art to the naked eye. Thus, there is a need for emission filters with high %T in a passband and little, or no, transmission elsewhere. Furthermore, the filters may need to have a relatively narrow passband. Better performance in these parameters, along with the right characteristics in the detector and microscope, makes the mapping of multicolored images easier.
With regard to other components for multicolor imaging, the objectives need to be color corrected. If they are not, then the signal and image registration for a given set of colors may be adversely impacted. This need for a more uniform – at least well understood – spectral response becomes more important as more colors are used.
Read our White Paper on Fluorescent Proteins: Theory, Applications, and Best Practices.